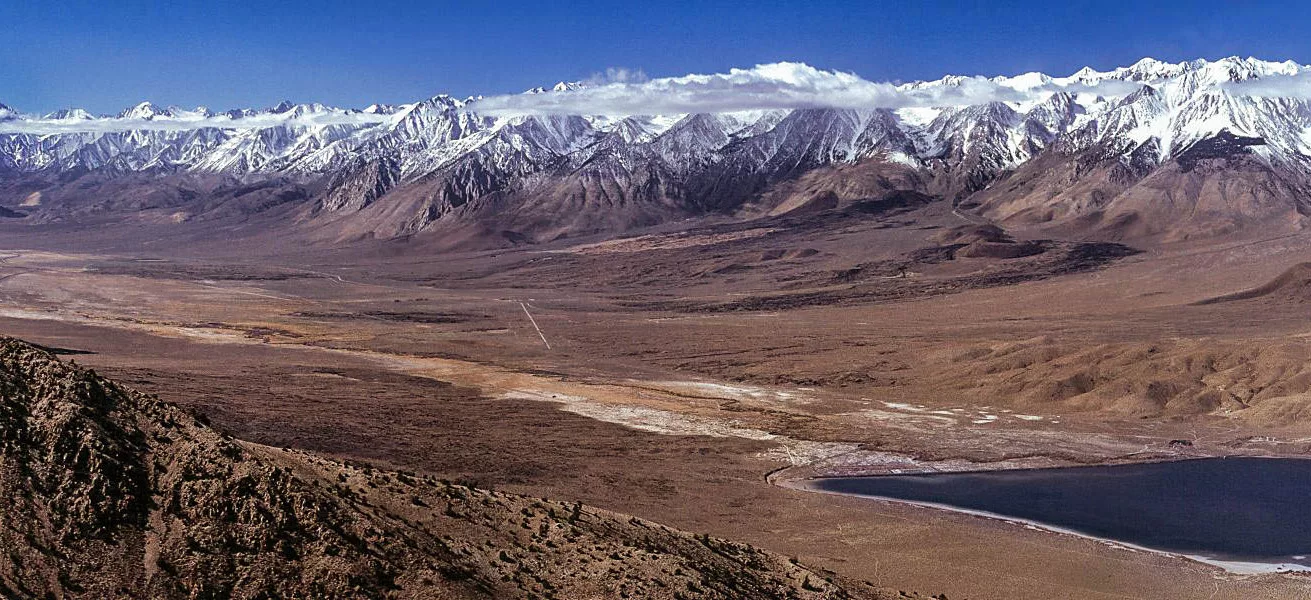
The western U.S. is slowly tearing apart. But the locus of this continental rending isn’t restricted to the San Andreas Fault that, in the U.S., separates a sliver of California from the rest. Everything west of the Rockies has been stretching over tens of millions of years. The result is that much of the west—the Basin and Range province, so named for linear mountain ranges separated by sediment-filled troughs—is tectonically and seismically active. Satellites detect faint but continuous movement imperceptible to most, and earthquakes occasionally rattle cities in states like Nevada and Utah.
In a new study published in Nature Communications, a team of scientists led by Lijun Liu, of the Chinese Academy of Sciences, used 3D geodynamic modeling to compute both lithospheric and mantle dynamics in the western U.S. The team reproduced direct observations of Earth’s surface via simulating what might be happening in the interior. They found that the structure of the lithosphere plays a critical role in governing deformation at a distance from plate boundaries. Moreover, they determined relative contributions of different categories of force—far-field, local, or basal.
Dividing the west
A tectonic plate comprises a piece of Earth’s lithosphere—crust and upper mantle separated by the Mohorovičić discontinuity, or Moho. Each plate slides along the mechanically weak asthenosphere. Most earthquakes and deformation concentrate along plate boundaries, but that’s not always the case. Scientists refer to deformation and earthquakes that occur far from the edges of a tectonic plate as “intracontinental” or “intraplate.”
Scientists often broadly divide western North America into tectonic provinces characterized by their lithospheric thickness, GPS-measured plate motions, or seismicity. In this study, the lithospheric thickness is based on a combination of seismic tomography and LITHO1.0, said Liu. (LITHO1.0 is a model that uses surface-wave data to estimate lithospheric thickness.) Surface motions derived from GPS come from the NSF GAGE archive operated by EarthScope. Earthquake information comes from the Global CMT catalog, which utilizes data from the Global Seismographic Network.
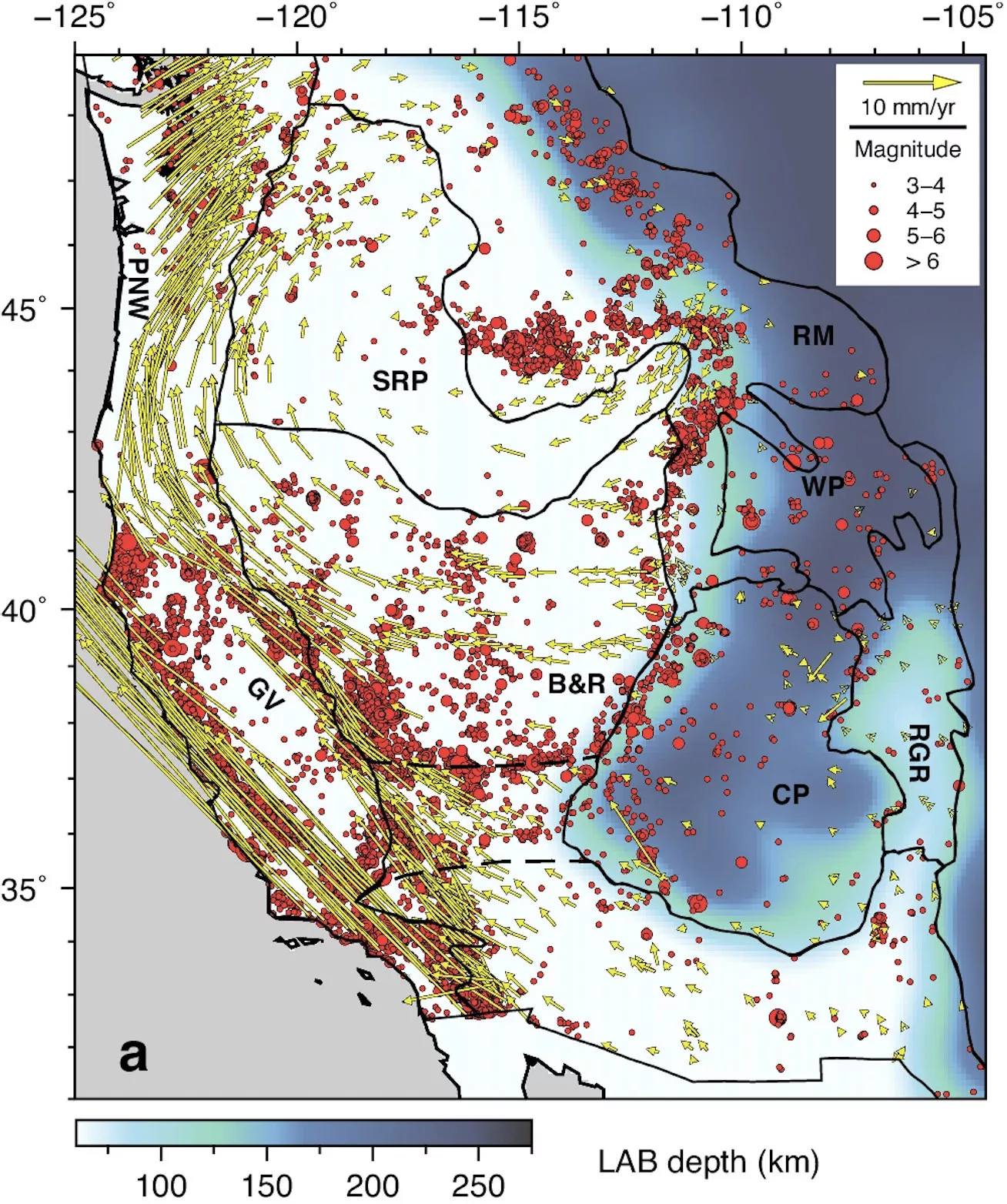
In the middle of North America, the Colorado Plateau forms a thick, seismically quiescent block that doesn’t move much relative to other regions. The Rio Grande Rift, which lies to the east, features far thinner lithosphere that’s experienced extension.
North of the Colorado Plateau, the Wyoming Plateau may be an even thicker chunk of lithosphere, Liu explained. The somewhat thinner Rocky Mountain region, northeast of the Colorado Plateau, has a considerably lithe western edge that coincides with the earthquake-prone Intermountain Seismic Belt. This swath of seismicity swings around a boot-shaped part of the Snake River Plain. The boot is the Yellowstone hotspot track, which looks like it’s kicking the Rocky Mountain region.
The rest of the Snake River Plain province features relatively thin lithosphere with diffuse plate motion and seismicity. To the south, the Basin and Range is far more seismically active, particularly along its western edge—Walker Lane. The steep eastern escarpment of California’s towering Sierra Nevada bounds the province to the west.
Beyond the Basin and Range, California’s Great Valley, seismically sleepy and lacking in deformation, is flanked by earthquakes and northwesterly plate motions of both Walker Lane to the east and the San Andreas to the west.
North of the Great Valley and west of the Snake River Plain is the Pacific Northwest, where eastward Cascadia subduction of the Juan de Fuca plate dominates seismicity patterns. Here, North America moves northeast, with the transition between San Andreas and subduction marked by a curve in the arrows that denote plate motion. These varied plate motions mean North America looks like it’s rotating clockwise, with the Great Valley and Colorado Plateau remaining relatively undeformed.
Possible plate forces
Possible drivers for intracontinental deformation fall into three broad categories: effects from distant plate boundaries (far-field), forces within the plate itself (local), and effects from below as the convecting mantle interacts with the plate’s underside (basal). Though researchers recognize that some combination of these forces must explain the geology and tectonics of the western U.S., the relative importance of each mechanism is controversial.
Long-range plate boundary effects reference what’s happening at the margins. If what’s happening at the edge of North America dictates intracontinental deformation, then San Andreas strike-slip motion and Cascadia subduction would be far-field culprits of Basin and Range extension and the diffuse motion of the Snake River Plane, respectively.
A more local driver—lithospheric body force—is governed by crustal thickness. High mountains and plateaus have high gravitational potential energy. Imagine dropping a ball from your hand to the ground versus off the edge of a skyscraper. The ball’s potential energy from the height of the skyscraper is greater than when it’s only a few feet above the ground. So, the thickest, highest parts of the U.S.—the Colorado Plateau and the Wyoming Plateau—command more gravitational potential energy than the thinner, lower-elevation regions to the west. If lithospheric body forces dominate, then the thick assemblage of rock would drive westward extension; in other words, local forces could matter more than far-field plate interactions.
Finally, below the lithosphere, the asthenosphere flows as the mantle convects, exerting drag forces on the plate’s underbelly. The shape—flat or undulatory—of the boundary between the lithosphere and asthenosphere could influence basal interactions, with a wavy underside possibly providing a stronger coupling between the plate and the asthenosphere.
Models in 3D
In this study, the team presents a set of ten 3D dynamic simulations, each of which models 40 million years of detailed lithospheric and convective mantle processes. These simulations incorporate coupling between the convecting mantle and lithosphere by including realistic structures uncovered in previous geophysical studies, although Liu points out that some models weaken the coupling to show the effect of this factor. Structures include the Juan de Fuca slab that’s subducting beneath northern California, Oregon and Washington, and the fully subducted Farallon plate, currently located beneath the central and eastern U.S. These slabs drive eastward upper mantle flow. On the other hand, thick lithosphere, like that of the Colorado Plateau, diverts mantle movement. The Yellowstone plume, said Liu, “is in the convective part of the model, although its mechanical effect is only a minor component in driving the horizontal flow. A plume generates mostly local vertical flow.”
All simulations include plate boundary forces because they represent the integrated effect of global mantle flow. In practice, this North America-centered study uses the motion of the surrounding tectonic plates as boundary conditions to mimic the far-field effects, Liu explained.
The team modeled the basal forces—the effect of mantle convection beneath a tectonic plate—in two ways. In some simulations, the boundary between the lithosphere and asthenosphere is wavy, as seismology reveals, whereas in others, it is flat as per previous assumptions.
Some simulations did not vary the depth to the Moho, which means that lateral variations in gravitational potential energy weren’t always included. In fact, capturing the effect of fine-scale gravitational potential energy involved greater complexity than simply varying the depth to the Moho. “Another important contributor,” said Liu, is “the depth to the lithosphere-asthenosphere boundary. Both factors are considered in the study.”
A synthesis
No single driving force can explain all surface observations, which include topography, seismicity, and crustal motions. The preferred simulation that best reproduced surface observations incorporated gravitational potential energy by varying depths to the Moho and the boundary between the lithosphere and asthenosphere; the latter varied laterally as well. The simulation also included lithospheric structures derived from other geophysics studies. The result was especially successful in reproducing observed deformation rates in Walker Lane and the Intermountain Seismic Belt.
In detail, each province appears to be varyingly affected by the three driving mechanisms. For instance, though all three drivers contribute to the stress field of the Pacific Northwest and part of the Snake River Plain, the forces acting on the underside of the plate (basal forces) dominate. Specifically, oceanic plate subduction acts on the underside of the North American plate, driving eastward shearing.
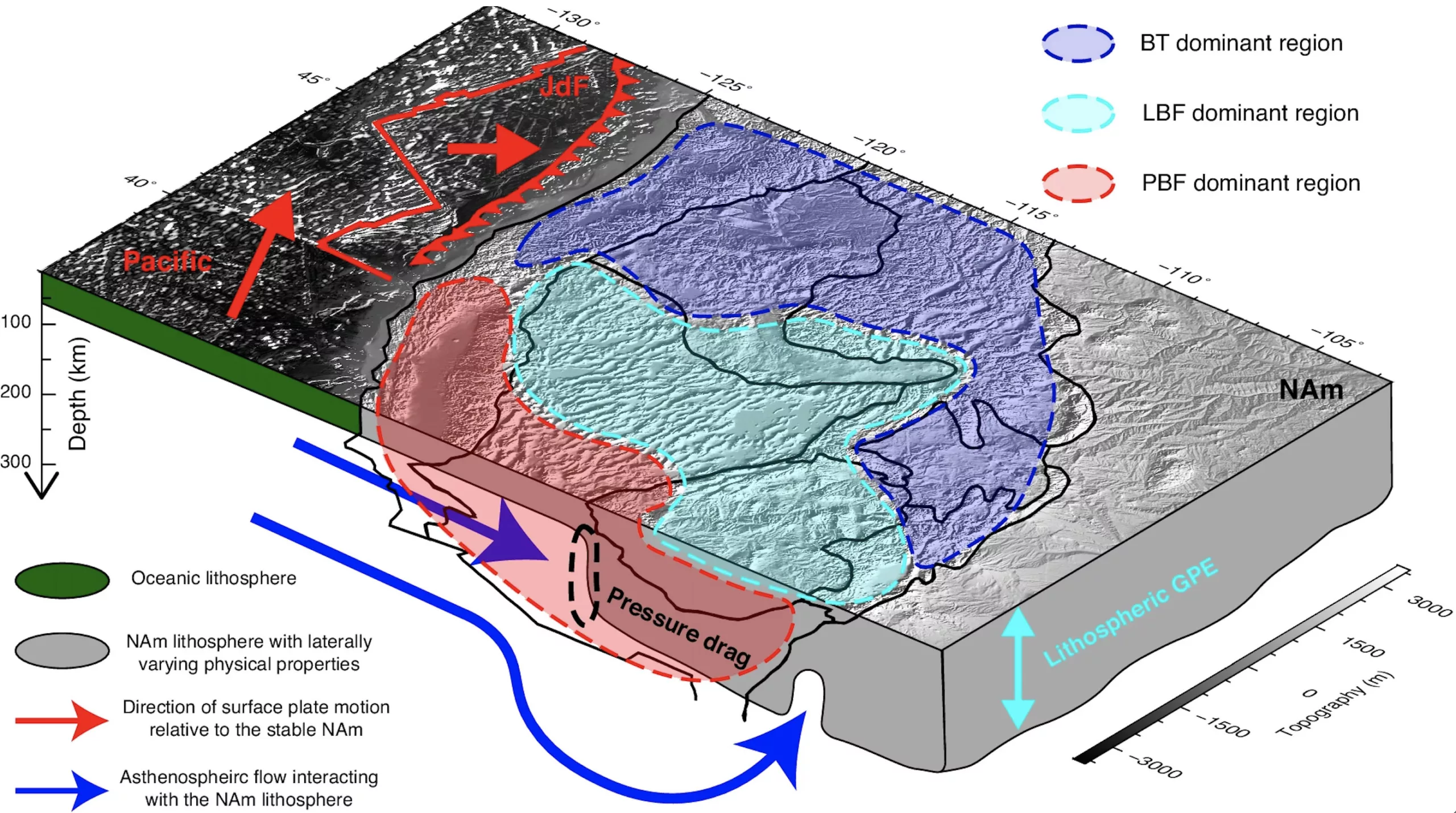
Westward extension in the central and southern Basin and Range appears to be driven by plate boundary effects (far-field forces) originating from the Pacific-North American boundary. However, both local gravitational potential energy and convective mantle forces beneath the lithosphere modify the motion, nudging extension into its western direction without any northward component.
On the eastern side of the study area, lithospheric structure plays a crucial role, thanks to a step change in lithospheric thickness between the eastern boundary of the Basin and Range and the thick Colorado and Wyoming Plateaus. This step, the authors say, localizes intracontinental deformation by forcing hot asthenosphere to interact with the deep keel of the thick plateaus, explaining the existence of the many intraplate earthquakes associated with the Intermountain Seismic Belt.
“This study highlights the complex nature of intraplate deformation,” said Liu, “and the necessity of using realistic data-driven physical models to solve these problems.”