The techniques in our geophysical toolkit have grown exponentially over the years. At the 2024 NSF SAGE/GAGE Community Science Workshop, many of the different sessions featured research conducted with the help of different kinds of revolutionary methods, including multiple methods of sensing events like ground deformation and moisture content. The NSF SAGE and GAGE Facilities operated by EarthScope Consortium support a variety of projects that use a variety of different tools and instruments. By both supporting and undertaking research in these fields, we can see the advancements and applications to geophysics and bettering society.
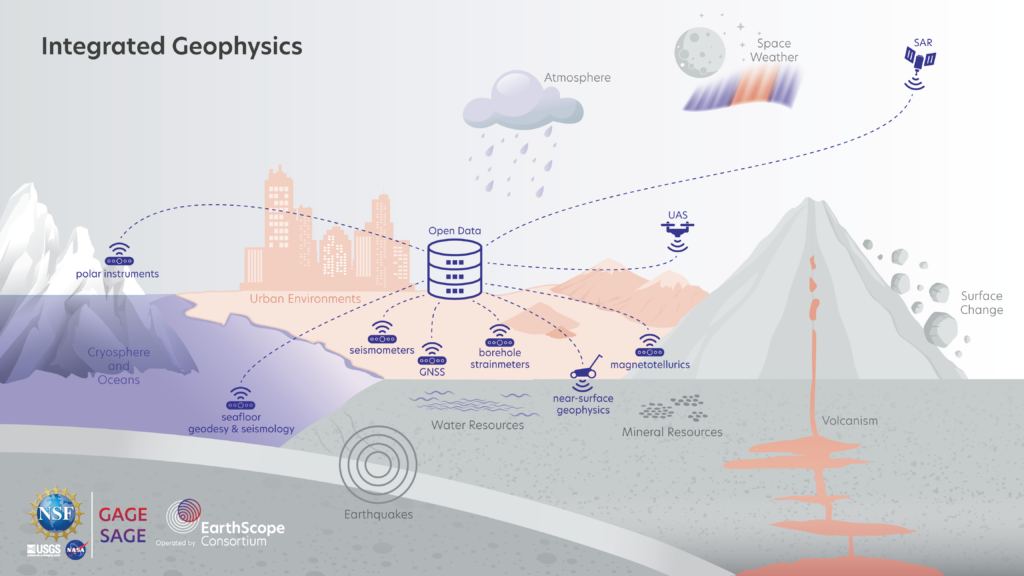
Many people who are not familiar with geophysical tools are probably already using it daily. The Global Positioning System (GPS), or more generally, the Global Navigation Satellite Systems (GNSS), is the satellite-based navigation we use in our cars and phones, and is an example. The receiver, in this case your phone or car, receives signals from the satellites in space and can measure its distance from them, pinpointing your exact location on Earth. But GNSS isn’t only used to help us navigate to the grocery store. It can also be used to monitor the locations of natural hazards, like moving landslides, and track motion along Earth’s plate boundaries.
Rebecca Kramer, a geophysicist at the Cascades Volcano Observatory, conducts research monitoring volcanic hazards using GNSS monitoring stations at major volcanic centers in Oregon and Washington. Despite harsh weather conditions and dense tree coverage hindering signals, Kramer’s team is dedicated to installing and maintaining stations in order to capture changes in ground deformation that could be related to volcanic processes. This monitoring is important for sensing patterns relating to volcanic activity and providing advanced warning to people in the area. This geophysical tool is one that has the ability to uncover these processes by detecting incredibly subtle surface changes and ground deformations to provide crucial insight into incoming volcanic activity.
GNSS-acoustic ranging (GNSS-A) is a way of pinpointing locations on the seafloor to help monitor its movements using similar processes as standard GNSS. Transponders are placed in known locations on the seafloor, but their signal can’t reach all the way to the satellites in space, since their signals can only travel through water. Instead, a sea surface platform, usually a ship or buoy, “translates” those signals into ones that can travel through air to the GNSS satellites. If the transponders change in location due to occurrences like tectonic movement, slow slip events, or slow earthquake ruptures on the seafloor, it will be shown in the signals. Noel Jackson, an Assistant Professor at the University of Kansas, conducts research on slow slip events, primarily by using GNSS-A. They are able to use this method to pinpoint exactly where slip is happening due to movements sensed by the transponders, and from that they can also hypothesize when and where onshore slip will occur as well.
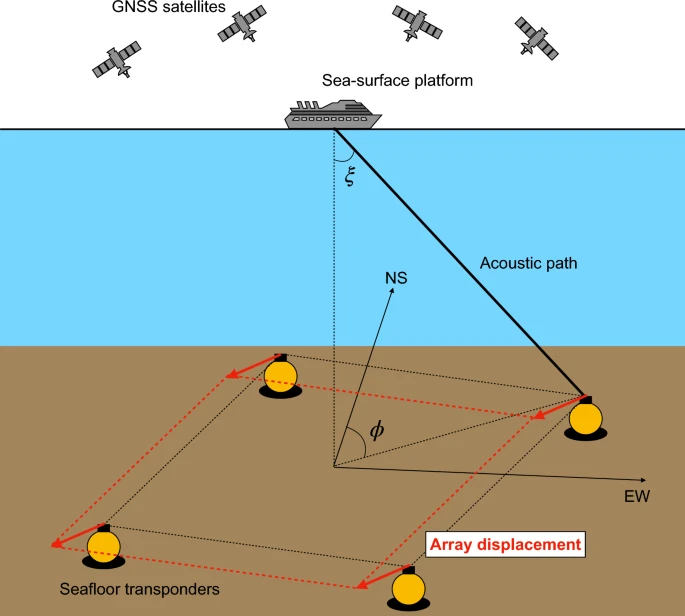
Credit: Fumiaki Tomita & Motoyuki Kido/Earth, Planets and Space
GNSS measures distance via transmission of radio waves between satellites and receivers, but things we measure can emit sound waves as well. The source of the sound may be moving, and in these cases, a couple transponders or hydrophones won’t be able to pick up its signal for an extended period of time over a longer distance. In these cases, we need a method of sensing that is longer than a single point—more spatially continuous. For these reasons, Distributed Acoustic Sensing (DAS) has become a very popular and essential way of collecting high-resolution data in a wide variety of situations. DAS utilizes a long, fiber optic cable that acts like a bunch of microphones listening to the environment for a disruption. The cable is connected to an interrogator, which sends light pulses through it. When there’s a strain (anything out of the ordinary that disrupts the light pulse), it sends a backscatter of the light to the interrogator to be analyzed. Using that data, researchers can determine the location of that source, its frequency, duration, and other features about it. Then, they can determine what the source is, whether it’s a whale call or an earthquake rupturing the seafloor.
Brad Lipovsky at the University of Washington and his team use DAS to monitor earthquake detection and ground motion hazard characterization, specifically helping to understand seismicity. They are able to use optical fiber sensor technology to make high resolution measurements of active deformation processes in areas like glaciers in Greenland and the Antarctic ice sheet. Lipovsky discussed the data his team collected from a fiber optic cable near Mt. Rainier, an active stratovolcano, in Washington state using DAS technology. This stratovolcano is important to study and understand due to its proximity to the metropolitan area, so understanding and predicting seismic signals is important for tracking patterns in the region.
Because DAS is sensitive to any changes in the environment, it can also track temperature changes around it. Yan Yang, a PhD student at Caltech, uses it for this purpose, repurposing old fibers for their own research. Their research uses DAS to monitor the temperature of the ground in places with varied climate conditions. The cables can “effectively capture responses to precipitation and droughts,” according to Yang. They also look at conditions at the South Pole using DAS to track density of the fresh snow layers, called firn, based on temperature, telling us about ice-sheet mass balance, hydrology, and paleoclimate of these areas.
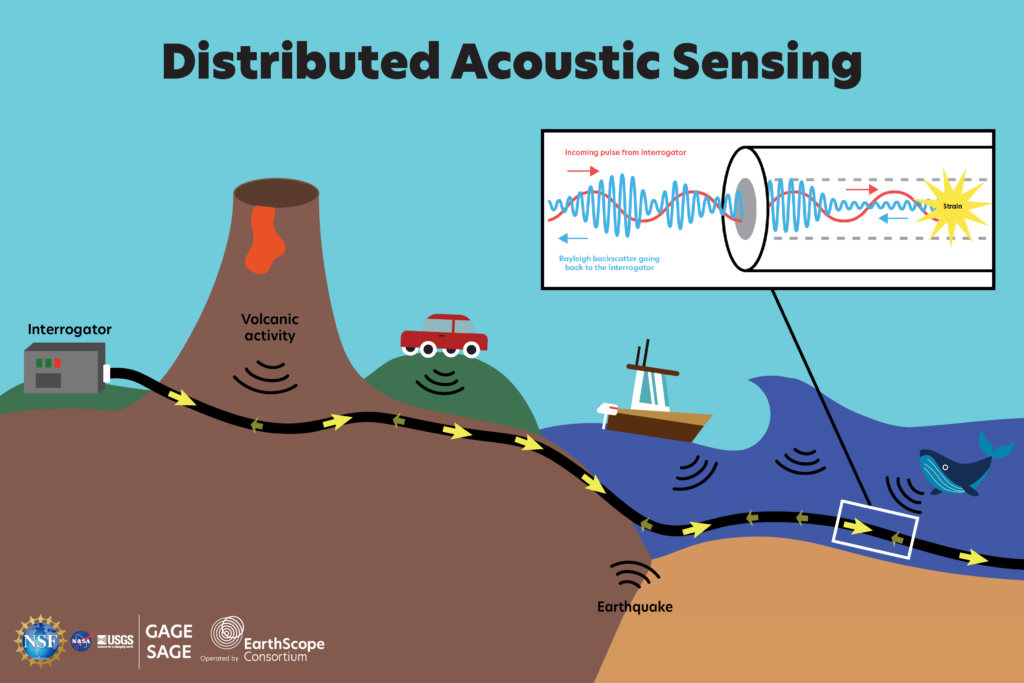
In many cases, however, like that of larger land areas or glaciers nearly a hundred miles long, we need a much larger scale technology to map surface changes. Interferometric Synthetic Aperture Radar (InSAR) is relied upon for measuring elevation changes in an area over time. InSAR uses multiple SAR images showing land elevations across an area to create maps of how the ground surface has deformed over time. These usually use satellites to send radar pulses down to the surface. Based on how long the scattered pulse takes to return and the differences in phases, frequency, and amplitude—just like DAS—it can calculate the height of the surface it hit. After two or more passes of the satellites over the area, the SAR images are used to create an interferogram, or a map of the area. Based on the recorded height of all the points, they can show the difference in distances of the traveled satellite signals between the passes of the satellite. InSAR is incredibly important for mapping areas of ground deformation, like active volcanoes, and watching their changes over time. These monitored changes are essential for finding patterns and understanding natural hazards, which can be life-saving in densely populated areas.
For example, in 2017, the Mud Creek Landslide in Big Sur, California covered a portion of Highway 1. Luckily, its failure and large collapse onto the road didn’t have any fatalities, but the highway was out of use for nearly a year and a half. Since then, teams like the one led by Alexander Handwerger at NASA-JPL use InSAR to characterize nearly a decade of the sliding of Mud Creek before it rapidly accelerated in pace and failed. They identified that a huge presence of rain after years of drought contributed to the failure, and they modeled this stable to unstable transition of the motion. Groups like these use the information from multiple sensors to identify the location, timing, and size of the landslide years, and hopefully forecast things like this and help understand what contributed to failures and hazards that have already occurred so we can hopefully be prepared for them in the future.
Not only can researchers track larger, more obvious catastrophes like landslides, but they can also capture more slight ground deformation due to processes like groundwater depletion. To understand changes in water resources, we can use InSAR to look at ground deformation and estimate its relation to aquifer system storage changes. Through research by those like Ryan Smith at Colorado State University, we can understand and improve on groundwater models and provide knowledge that can be used for policies to conserve our water supply and make more sustainable efforts.
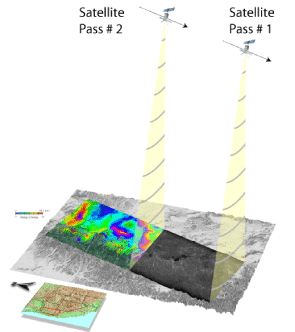
GNSS, DAS, and InSAR are just three examples of common types of geophysical techniques being used in the field today. By featuring work done with these techniques at the 2024 NSF SAGE/GAGE Community Science Workshop, others in the geoscience community can see the benefits of the science beyond the original user group. The more we can understand and model geophysical events around us, the better we can prepare ourselves and work with the teams conducting research using these techniques to create a better society and a more resilient community.